
Control of Particle Size by Feed Composition in the Nanolatexes (2014)
.pdf
charge. Monomer droplets feed particles with fresh monomers thus causing particles to grow at a rate which is thermodynamically kept at a constant level. This led to the formation of particles as large as 68 and 83 nm in diameter for MMA/St and VA/BA systems, respectively. We find from Fig. 5b and 5f that the presence of relatively small amount of monomer droplets in the initial charge of reactor can decrease the number of particles by an order of magnitude. With (R/F)M = 0, no free monomer droplets existed in the system and thus, the rate of particle growth was controlled by the rate of monomer addition right from the beginning [47]. This led to the formation of a large number of small particles.
For the conventional MMA/St and VA/BA semicontinuous systems, R/F)M > 0%, there were initially free monomer droplets in the reaction medium and as a result, the rate of reactions was higher than when polymerization started under starved conditions. However, a very large Np obtained under starved conditions (see Fig. 5b) resulted in the rate of VA/BA reaction overtaking that with (R/F)M = 10% after some time into the reaction and achieving a higher final conversion. The same trend was about to emerge for MMA/St system with (R/F)M = 20% too (Fig. 5e), but the rate of reaction increased at higher conversions due to the gel effect, which is likely to occur if PMMA/PSt particles are larger than 50 nm in diameter [19].
The polydispersity index of polymer particles at the end of nucleation, judged by the time that Np becomes constant with time, was 1.04 and 1.06 for MMA/St and VA/BA nanolatexes made via monomer-starved semicontinuous copolymerizations, but rose to 1.11 and 1.12 for latexes made via the conventional semicontinuous polymerizations, respectively, indicating narrower distributions for nanolatexes. Monomer-starved nucleation is known to produce narrow PSDs [16].
Copolymer composition drifts versus time are shown in Fig.5c and 5g. For both systems, the early copolymers were richer in the least water-soluble and most reactive monomer, namely BA and St, but later the copolymers were more homogenous in composition. We found that composition drift was significantly less for monomer-starved semicontinuous polymerization because of high instantaneous conversions achieved in the course of reaction. The copolymer compositions of nanolatexes made with different feed compositions but under the conditions of (R/F)M
= 0, as described in section 3.1, were also found to be very close to the comonomer feed compositions used. This suggests that the composition drift in copolymer nanolatexes is less significant than their counterpart latexes made by conventional methods under similar conditions (i.e., the same rate of monomer addition).
For the conventional semicontinuous runs with (R/F)M > 0%, the surface tension increased abruptly, shortly after the start of reaction because of a high rate of growth for particles, in the presence of monomer droplets, that shortens the nucleation time. For the emulsion runs, (R/F)M = 0, the surface tension remained close to that of CMC during nucleation. It then showed a rather steep increase after the end of nucleation, which can be inferred from Fig. 5b and 5f where Np reaches a constant value, and finally overtook those of conventional runs (see Fig. 5d and 5h). This latter is simply because of the large particle surface areas generated with (R/F)M = 0, which adsorb surfactant molecules from the aqueous phase and causes the surface tension to rise.
Partially water-soluble monomers such as VA and MMA can serve to maintain nucleation under starved conditions, by being dissolved in the water phase, if the rate of reaction is not significantly suppressed by their lower concentration in particles. However, aqueous-phase polymerization can occur for a comonomer which is partially
10

soluble in water. From one hand, aqueous-phase polymerization can lead to the formation of a copolymer which is enriched in the water-soluble monomer. From the other hand, the favored distribution of water-soluble monomers (i.e., MMA and VA) in the water phase leaves a more St-rich or BA-rich monomer composition in the polymer particles, where polymerization occurs, leading to the enrichment of copolymer in less water-soluble monomers. The combined effect is a more drastic composition drift, particularly during the early (seeding) stage of the conventional semicontinuous process where there exists an excess amount of monomers (i.e, monomer-flooded system).
Assuming that the solubility of the pair of monomers in the water phase is not mutually (and substantially) affected by their presence, an estimation of composition drift in the conventional semicontinuous polymerizations with the initial monomer charge can be presented as follows. VA and MMA solubility in water is around 2.5 and 1.5 wt %, respectively, whereas BA and St solubility in water is very low (around 0.1 wt%). If the solubility of VA in water is taken into account (for the VA/BA = 61/39 system with the initial charge of 10%, 12.5 g of VA monomer is dissolved in the water phase, see Table 1), a monomer droplet phase (or particle phase) with composition of VA/BA (molar) = 30/70 will form at the early stage of polymerization when the volume of polymer phase is still not significant (practically at zero conversion). This feed composition theoretically (see any polymer textbook for copolymer composition equation) should produce a copolymer with the instantaneous feed composition of FBA= 0.94 at time zero. Fig. 5g clearly shows a value around FBA = 0.75 at t ≈ 0, indicating strong contribution from aqueous-phase polymerization of VA, which is in agreement with the literature [48]. For MMA/St = 50/50 system with 20% initial monomer charge (see Table 1), 9 g of the initial MMA is dissolved in water, giving a droplet molar phase ratio of MMA/St = 30/70. This will produce a copolymer with FSt = 0.65 at time zero, which is only marginally larger than the first experimental point value shown in Fig. 5c. This suggests a negligible aqueous-phase polymerization for MMA/St system. Nomura et al. stated that water-soluble polymerization is usually associated with homogenous nucleation occurring in polymerization systems with low surfactant concentrations. They did not observe any aqueous phase polymerization for MMA/St system when the surfactant concentration was high (i.e., above CMC) [49], similar to the current study.
We found from the results that the composition drift can be significant for the conventional semicontinuous process using an initial comonomer charge. We believe the composition drift for conventional semicontinuous polymerizations is even more significant than what can be detected by gas chromatography, as shown in Fig. 5, because the technique cannot differentiate the sequence of monomers in the polymer chains (i.e., resulting from homoor block copolymerization reactions occurring within particles and in the water phase because of monomer partitioning). For the starved nucleation, these effects are less significant because there is no free monomer droplets in the reaction medium, the instantaneous conversion in the particles is relatively high and as a result the concentration of monomers in the water phase is relatively low. The comparison of the time evolutions of Np and F clearly shows that particle nucleation in the conventional semicontinuous process occurred during the time that composition drift was significant. This is the reason why conventional semicontinuous polymerization systems cannot reproduce a Np-f behavior similar to that reported in this work for monomer-starved semicontinuous emulsion polymerizations.
11

DISCUSSION
The main three parameters affecting the number of particles, which might vary with copolymerization conditions, are the volumetric rate of particle growth ( ), the rate of radical entry into micelles and particles (Ri), and the surfactant parking area (as).
Particle growth: For a binary batch emulsion copolymerization, the rate of growth for a particle containing single radical, , is a complex function of the comonomers reactivity and concentrations, as given by [2]
|
3 |
|
m |
m |
kp11kp22 (r1 f12 2 f1 f2 |
r2 |
f22 ) |
(3) |
4 NA |
|
p |
p |
kp22r1 f1 kp11r2 |
f2 |
|
||
|
|
|
where m and p are the average density of monomers and copolymer, respectively, m and p are volume fraction of monomers and polymer in the polymer particles at equilibrium conditions, respectively, and kp11 and kp22 are the propagation rate constants for radicals of types 1 and 2 reacting with similar monomers, respectively. Using appropriate kinetic data for Eq.3, the variations in with feed composition were calculated and shown in Fig. 6.
Fig. 6. Variations in l/s) with fMMA and fVA in MMA/St and VA/BA emulsion copolymerization, respectively. PS
= 1.044, PBA = 1.04, PVA = 1.11, and PMMA = 1.178 g/cm3; S = 0.88, BA = 0.869, VA = 0.893, and MMA = 0.94 g/cm3; rVA= 0.08, rBA=7.20, rSt = 0.52 and rMMA = 0.46; kpVA =4000, kpBA = 200, kpSt = 210, and kpMMA = 650
l.mol.s-1; T = 50○C [28,41]. We used p=f1 p1+f2 p2 and m=f1 m1+f2 m2, and for simplicity assumed m = p = 0.50.
The number of particles in a typical batch emulsion polymerization of sparingly water-soluble monomers can be estimated by Np k2 (as NA [S])0.60 (Ri / )0.40 , suggesting that Np increases with decreasing rate of particle growth ( ).
Assuming that the same equation can roughly represent the systems under study, it is clearly seen from Fig. 6 that increases with fMMA in MMA/St copolymerization system, implying that MMA monomer contributes to a decrease in Np by its virtue of high propagation (growth) rate. For VA/BA system, VA serves to decrease the rate of growth of particles and hence contribute to an increase in Np. But this trend breaks down at high values of fVA where a minimum occurs around fVA = 0.90, implying an opposite trend thereafter. For a monomer-starved polymerization when Ra/Np << , the rate of growth of particles is controlled by the rate of monomer addition; ≈ Ra/Np. This implies that the rate of growth of particles is almost independent of the kinetics of polymerization (i.e. feed composition) and is governed by the rate of monomer addition; Rp≈ Ra. As a result the comonomer composition in
12
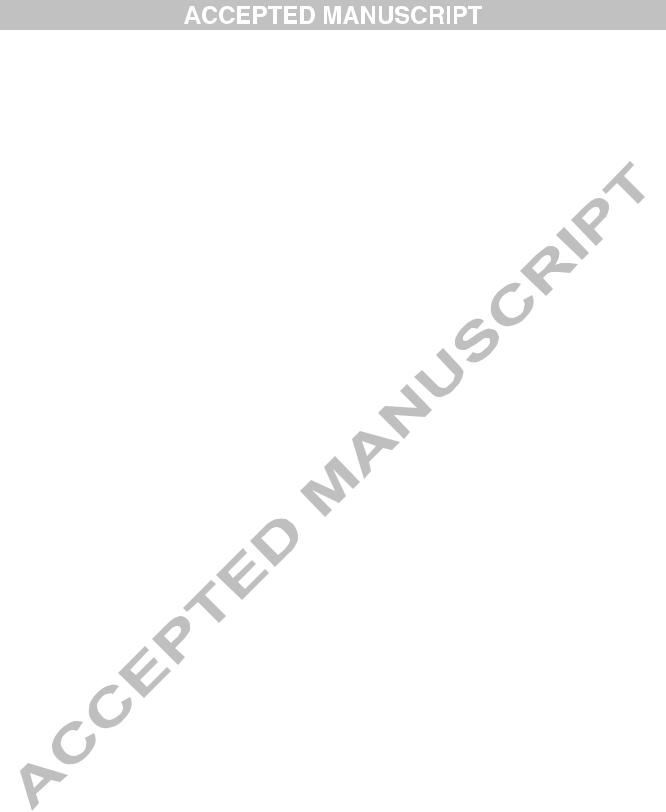
the feed and copolymer becomes comparable; f1= F1. At high conversions, usually chain transfer to polymer is a possible fate for propagating radicals of some monomers such as BA and VA, but neither affects the rate of particle growth nor nucleation under the condition of Rp= Ra. Alternatively, the rate of desorption of radicals in a highly monomer-starved semicontinuous emulsion copolymerization, if any, and their subsequent termination in the water phase would not significantly affect the rate of particle growth if Rp= Ra.
Radical entry into micelles: Before a radical can enter a micelle, it has to propagate with monomers dissolved in the water phase to reach a size that can be adsorbed by micelles. Many of such radicals will terminate in the water phase, depending on polymerization conditions, before they can enter micelles or particles. In a typical emulsion polymerization, the rate of radical generation in the aqueous phase, Ri, varies with the efficiency of radical entry as given by;
Ri 2 kd [I ] |
(4) |
where is the initiator efficiency (shown by this character rather than f to avoid confusion) which is a complex function of initiator decomposition, monomers solubility and their rate of propagation and termination in water, the thermodynamic properties of the resulting oligomers in the water phase such as the critical chain length for entry.
In a typical homopolymerization, the entry efficiency is usually around 100% for water-soluble monomers such as MMA, but much lower for sparingly water-soluble monomers such as St. Oligomeric MMA or VA radicals formed in the aqueous phase can propagate quickly in the water phase to reach the critical size for entry before they terminate. This may suggest that the rate of radical entry in a copolymerization reaction is therefore governed by the most water-soluble monomer, and is independent of comonomer feed. However, this feature is significantly moderated by the presence of even small amount of the less water-soluble co-monomer in water via changing the rate of propagation and altering the critical chain length for entry into micelles. Nomura et al. indicated that in MMA/St copolymerization, a higher rate of radical entry into micelles, in comparison to that for St, should be considered. They showed that the efficiency of radical entry into micelles ( ) for MMA/St system can equally be represented by [28]:
1 f1 2 f2 |
(5) |
where fi represents monomer concentration in micelles, which has been assumed to be proportional to monomer concentration in the feed. Nomura et al. also claimed that almost all polymer particles in typical emulsion (co)polymerization of monomers such as MMA and VA are generated by the charged radicals stemming from the initiator, while monomeric radicals mostly terminate in the water phase [28], indicating Eq.5 can adequately represent nucleation process for these monomers. We adopt Eq.5, for the sake of simplicity for this discussion.
Surfactant parking area: Particle formation is affected by the rate at which surfactants are extracted from the water phase. As the concentration of water-soluble monomer in the copolymer feed increases, the average area occupied by a molecule of surfactant becomes larger. This will reduce the rate of depletion of emulsifier micelles and thus increases Np (in the absence of particle coagulation). A large parking area (as) would allow for a longer nucleation period. For copolymerization reactions, as represents the average adsorption area of the emulsifier molecule on the surface of copolymer particles and it is approximately correlated with the feed composition by
13

as F1s a1 F2s a2 f1 (a1 a2 ) a2 (6)
F1s and F2s are in fact the surface composition of polymer particles. For a homogenous copolymer system made via semicontinuous polymerization, these are equivalent to the corresponding composition in the feed. The typical values of a for PS, PBA, PMMA, and PVA particles are 0.43, 0.63, 0.80, and 1.10 nm2, respectively, which show a wide disparity among the monomers [6,24].
Particle nucleation: Particle formation in a typical semicontinuous emulsion homopolymerization of sparingly water-soluble monomers under monomer-starved conditions is given in eq.1. There are indications in the literature that Eq.1 can equally be applicable to more water-soluble monomers such as methyl methacrylate [4], while there are reports that do not find such agreements [6]. We assume that eq. 1, which is an extension of classical Smith and Evart nucleation theory, is adequate for estimation of Np in monomer-starved emulsion copolymerization of a pair of sparingly water-soluble monomer and monomer with a moderate water solubility [35]. This equation can theoretically be adapted to a monomer-starved emulsion copolymerization reaction if modified for the rate of radical entry (Eqs. 4&5) and parking area (Eq.6), as follows:
N p k3 [a2 f1 (a1 a2 ))N A [S]][ 2 f1 ( 1 2 )]2 / 3 [I ]2 / 3 Ra 2 / 3 (7)
where the composition of the most water-soluble monomer has been shown by f1. Eq. 7 clearly shows that Np in copolymerization systems is a complex, but increasing function of the comonomer feed ratio of the more watersoluble monomer. Qualitatively, this prediction fits with the data presented in in Fig. 2 and 4.
We can simplify Eq.7 by assuming that the water-soluble monomer is in higher proportion than the other monomer f1 >> f2, and significantly modifies the surfactant parking area ( a1 a2 ) and the rate of radical entry ( 1 2 ) , as follows:
N p k4 f15 / 3[S][I ]2 / 3 Ra 2 / 3 |
(8) |
The above equation shows an explicit variations in Np with the feed composition (i.e., the fraction of the most watersoluble comonomer in the feed). The pattern, in which the experimental Np varies with feed compositions, as shown in Fig. 3, appears to be exponential, but for the sake of comparison, we can estimate it by power correlations across short intervals. Fig. 3 and 5 clearly show that the exponent continuously increases with f1 reaching a value around 1.67 in the middle, in line with the exponent of 5/3 indicated in Eq. 8, but approached values greater than 1.7 in the last quartile of f1. The latter is not surprising because of simplifications made in derivation of Eq.8 and also that water-soluble monomers often show a greater dependence on the rate of monomer addition than the styrenic monomers [6]. We conclude that Np in batch or conventional semicontinuous copolymerization, in which a part of reaction is carried out batchwise, cannot be easily correlated with the feed composition because of the significant variations in , surfactant coverage and initiation rate with feed composition.
14

CONCLUSION
The growth of polymer particles in emulsion copolymerization is a function of the reactivity of the monomers involved as well as comonomers concentrations. This causes the dynamic of the system continuously changes with time due composition drift in all phases, leading to inconsistent variations in latex properties with comonomer feed composition [27-36]. Preparation of nanolatexes by monomer-starved semicontinuous emulsion polymerization has recently been the focus of many research in the field of polymeric nanoparticles [1-7], but its extension to copolymer nanoparticles has been scarce [8]. In this research, we produced copolymer nanolatexes by semicontinuous monomer-starved emulsion copolymerization and unraveled their interesting features including; their size is inversely related to the ratio of the most hydrophilic monomer in the feed, they achieve a high conversion because of large number of small particles produced, and for the same reason are more homogenous in composition than their latex counterparts from conventional copolymerization systems carried out under similar conditions.
References
[1]J.S. Nunes, J.M. Asua, Langmuir 29 (2013) 3895.
[2]Y. Chen, F. Jahanzad, S. Sajjadi, Langmuir 29 (2013) 5650.
[3]D. England, N. Tambe, J. Texter, Macro Lett. 1 (2012) 310.
[4]J.S. Nunes, J.M. Asua, Langmuir 28 (2012) 7333.
[5]N.M.B. Smeets, R.P. Moraes, J.A. Wood, T.F.L. McKenna, Langmuir 27 (2011) 575.
[6]S. Sajjadi, Langmuir 23 (2007) 1018.
[7] L. Boguslavsky, S. Baruch, S. Margel, J. Colloid Interface Sci. 289 (2005) 71. [8] H. Wang, Q. Pan, G.L. Rempel, Eurp. Polym. J. 47 (2011) 973.
[9] H. Wang, Q. Pan, M. Hammond, G.L. Rempel, J. Polym. Sci. Polym. Chem. Ed. 50 (2012) 736.
[10] M.G. Perez-Garcia, E.V. Torres, I. Ceja, L.A. Perez-Carrillo, R.G. Lopez, F. Lopez-Serrano, E. Mendizabal, J.E. Puig, J. Polym. Sci. Polym. Chem. Ed. (50) 2012 3332.
[11] C. Cannizzo, C.R. Mayer, F. Secheresse, C. Larpent, Adv. Materials 17 (2005) 2888.
[12]J.S. Guo, M.S. El-aasser, J.W. Vanderhoff, J. Polym. Sci. Part A-Polym. Chem. 27 (1989) 691.
[13]F. Bleger, A.K. Murthy, F. Pla, E.W. Kaler, Macromolecules 1994, 27, 2559-2565.
[14]M. Nomura, K. Suzuki, Macromol. Chem. and Phys. 198 (1997) 3025.
[15]N.M.B. Smeets, T.F.L. Mckenna, J. Colloid Interface Sci. 383 (2012) 28.
[16]S. Sajjadi, AICHE J. 55 (2005) 3191.
[17]L.M. Gan, N. Lian, C.H. Chew, G.Z. Li, Langmuir 10 (1994) 2197.
[18]S. Sajjadi, J. Polym. Sci. Polym. Chem. Ed. 39 (2001) 3940.
[19]G.W. He, Q. Pan, G.L. Rempel, Macromol. Rapid Commu. 24 (2003) 585.
[20]A.G. Ramirez, R.G. Lopez, K. Tauer, Macromolecules 37 (2004) 2738.
[21]S. Sajjadi, M. Yianneskis, Polym. React. Eng. 11 (2003) 715.
15

[22]N. Sosa, R.D. Peralta, R.G. Lopez, L.F. Ramos, I. Katime, C. Cesteros, J.E. Mendizabal, Polymer 42 (2001) 6923.
[23]K.D. Hermanson, E.W. Kaler, Macromolecules 36 (2003) 1836.
[24]F. Codari, D. Moscatelli, M. Furlan, M. Lattuada, M. Morbidelli, M. Soos, Langmuir 30 (2014) 2266.
[25]J.J. Krackler, H. Naidus, J. Polym. Sci. Part C. 27 (1969) 207.
[26]S. Sajjadi, Polymer 44 (2003) 223.
[27]D.O. Shah, Micelles, microemulsions and monolayers; Science and Technology, 1998, Dekker, New York.
[28]C.S. Chern, L.J. Wu, J. Polym. Sci. Polym. Chem. Ed. 39 (2001) 3199.
[29]L.M. Gan, C.H. Chew, I. Lye, L. Ma, G. Li Polymer 34 (1993) 3860.
[30]N. Sutterlin, Influence of monomer polarity on particle formation in emulsion polymerization, in “Polymer Colloids II”, R. M. Fitch (Ed), 1981, plenum press, New York, 583-597.
[31]S. Sajjadi, F. Jahanzad, Chem. Eng. Sci. 61 (2006) 3001.
[32]F. Jahanzad, J. Appl. Polym. Sci.117 (2010) 84.
[33]K.C. Lee, M.S. El-AAsser, W. Vanderhoff, J. Appl. Polym. Sci. 45 (1992) 2207.
[34]M. Nomura, I. Horie, M. Kubo, K. Fujita, J. Appl. Polym. Sci. 37 (1989) 1029.
[35]J. Forcada, J.M. Asua, J. Poly. Sci. Polym. Chem. Ed. 28 (1990) 987.
[36]K. Ouzineb, M.F. Heredia, C. Graillat, T.F. McKenna, J. Polym. Sci. Polym. Chem. Ed. 39 (2001) 2832.
[37]X.Z. Kong, C. Pichot, J. Guillot, Colloid and Polym. Sci. 165 (1987) 791.
[38]M.S. El-Aasser T. Macgawinata J.W. Vanderhoff, J. Polym. Sci. Polym. Chem. 21 (1983) 2363.
[39]H. Ahmad, A.H.M.T. Islam, M.A. Hossain, M.A.J. Miah, K. Tauer, E-Polymer 030 (2006) 1.
[40]C.S. Chern, H. Hsu, J. Appl. Polym. Sci. 55 (1995) 571.
[41]P. Canu, S. Canegallo, M. Morbidelli, G. Storti, J. Appl. Polym. Sci. 54 (1994) 1899.
[42]G. Arzamendi, J.M. Asua, J. Appl. Polym. Sci. 38 (1989) 2019.
[43]S. Roy, S. Devi, Polymer 38 (1997) 3325.
[44]V.M Ovando-Medina, R.D. Peralta, E. Mendizabal, Colloid and Polym. Sci. 2009, 287, 561-568.
[45]B. W. Brooks, Br. Polym. J. 3 (1971), 269.
[46]S. Sajjadi, J. Polym. Sci. Polym. Chem. Ed. 38 (2000) 3612.
[47]S. Sajjadi, B.W. Brooks, J. Appl. Polym. Sci. 74 (1999) 3094.
[48]X.Z. Kong, C. Pichot, J. Guillot, Eur. Polym. J. 24 (1988) 485.
[49]M. Nomura, U.S. Satpathy, Y. Kouno, K. Fujita, J. Polym. Sci. Polymer Chem. Let. 26 (1988) 385.
16