
Биоинженерия / ТИ_ССС / nm1394
.pdf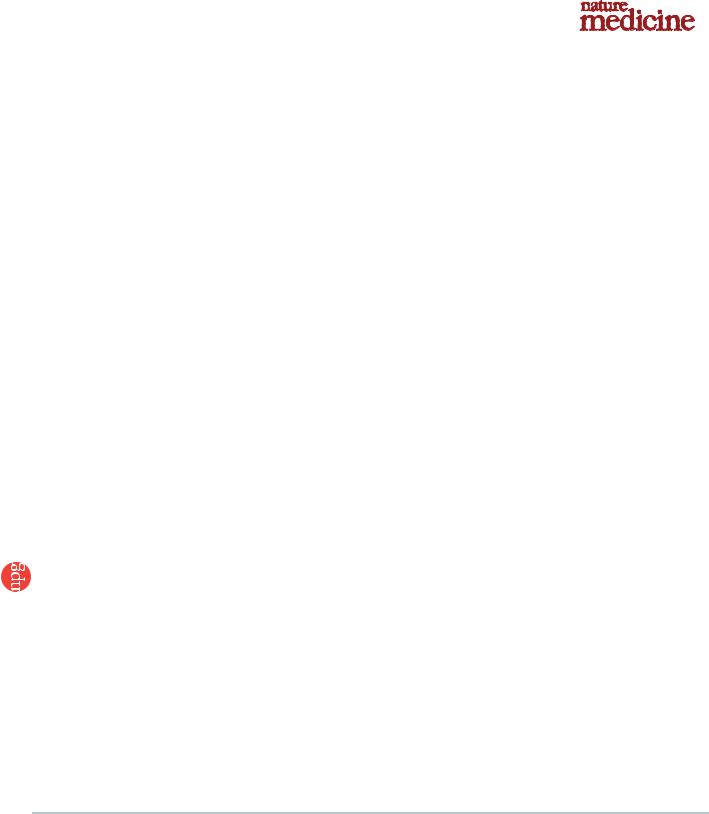
T E C H N I C A L R E P O R T S
© 2006 Nature Publishing Group http://www.nature.com/naturemedicine
Engineered heart tissue grafts improve systolic and diastolic function in infarcted rat hearts
Wolfram-Hubertus Zimmermann1,6, Ivan Melnychenko1,6, Gerald Wasmeier2, Michael Didie´1, Hiroshi Naito1, Uwe Nixdorff2, Andreas Hess3, Lubos Budinsky3, Kay Brune3, Bjela Michaelis4, Stefan Dhein4,
Alexander Schwoerer5, Heimo Ehmke5 & Thomas Eschenhagen1
The concept of regenerating diseased myocardium by implantation of tissue-engineered heart muscle is intriguing, but convincing evidence is lacking that heart tissues can be generated at a size and with contractile properties that would lend considerable support to failing hearts. Here we created large (thickness/diameter, 1–4 mm/15 mm), force-generating engineered heart tissue from neonatal rat heart cells. Engineered heart tissue formed thick cardiac muscle layers when implanted on myocardial infarcts in immune-suppressed rats. When evaluated 28 d later, engineered heart tissue showed undelayed electrical coupling to the native myocardium without evidence of arrhythmia induction. Moreover, engineered heart tissue prevented further dilation, induced systolic wall thickening of infarcted myocardial segments and improved fractional area shortening of infarcted hearts compared to controls (sham operation and noncontractile constructs). Thus, our study provides evidence that large contractile cardiac tissue grafts can be constructed in vitro, can survive after implantation and can support contractile function of infarcted hearts.
Endogenous regenerative mechanisms do not suffice to compensate for cardiomyocyte death after myocardial infarction. Pharmacotherapy can delay, but not reverse, the natural course of the disease. Thus, exogenous regenerative strategies have gained increasing attention1,2. Cells of various origins and developmental stages have been grafted into healthy and diseased hearts, including primary cells3–7, embryonic stem cell–derived cardiomyocytes8 and potential cardiac progenitors from peripheral blood9, bone marrow10–12 or heart13,14. Most studies have reported partial restoration of myocardial function in
various disease models7,9–12. The mechanisms of functional improvement are currently under debate15–17, and may include de novo
cardiogenesis from engrafted cells, paracrine effects or homing of cardiac progenitors.
A different concept in cardiac regeneration is grafting ex vivo engineered heart muscle. This approach may theoretically allow
complete replacement of diseased myocardium or reconstitution of cardiac malformations. However, cardiac tissue engineering is still in its infancy for several reasons. (i) Postnatal cardiomyocytes do not or do not sufficiently replicate. Thus, large-scale tissue engineering will require alternative cell sources. (ii) To repair myocardial infarctions or correct heart defects, heart tissue patches need to be engineered at a size and with contractile features that can lend considerable support to failing hearts. Size, as well as function and in vivo survival, depend crucially on metabolic supply. Thus, vascularization is a prerequisite for successful creation and survival of engrafted large cardiac tissue patches. (iii) The heart not only needs systolic strength, but depends on unimpeded diastolic relaxation. Consequently, engineered heart muscle must have a large compliance. (iv) Any successful tissue engineering concept will depend on structural and electrical integration of implanted tissue into the host myocardium. Although recent progress in stem cell research has opened new perspectives for cell sourcing (for example, from embryonic18 or adult stem cells13,19,20), the other conceptual challenges remain.
We have developed a methodology to create engineered heart tissue (EHT) from neonatal rat heart cells21–23. EHTs differ from classical scaffold-based tissue-engineered cardiac constructs24–26 because they are originally made from heart cells, liquid collagen I and Matrigel as well as growth supplements, reconstituted in circular molds and subjected to mechanical strain. Under these conditions, cardiac organoids develop spontaneously and show contractile as well as electrophysiological properties of working myocardium23. The first implantation experiments in healthy rats showed survival, strong vascularization and signs of terminal differentiation of EHT grafts27.
Here, we systematically evaluated the suitability of EHT in heart muscle repair after myocardial infarction. We took the following steps to define EHT-specific effects and minimize variability as well as observer bias: (i) control experiments included sham surgeries (no grafts) and implantation of different noncontractile grafts; (ii) all interventions were performed in rats with echocardiographically confirmed large transmural infarcts; (iii) functional effects of EHT
1Institute of Experimental and Clinical Pharmacology, University Medical Center Hamburg-Eppendorf, Martinistra e 52, 20246 Hamburg, Germany. 2Medical Clinic II, Friedrich-Alexander-University of Erlangen-Nuremberg, Ulmenweg 18, 91054 Erlangen, Germany. 3Institute of Experimental and Clinical Pharmacology and Toxicology, Friedrich-Alexander-University of Erlangen-Nuremberg, Fahrstra e 17, 91054 Erlangen, Germany. 4Heart Center, Department of Cardiac Surgery, University of Leipzig, Stru¨mpellstra e 39, 04289 Leipzig, Germany. 5Institute of Vegetative Physiology and Pathophysiology, University Medical Center Hamburg-Eppendorf, Martinistra e 52, 20246 Hamburg, Germany. 6These authors contributed equally to this work. Correspondence should be addressed to T.E. (t.eschenhagen@ uke.uni-hamburg.de) or W.-H.Z. (w.zimmermann@uke.uni-hamburg.de).
Received 7 December 2005; accepted 7 March 2006; published online 2 April 2006; doi:10.1038/nm1394
4 5 2 |
VOLUME 12 [ NUMBER 4 [ APRIL 2006 NATURE MEDICINE |
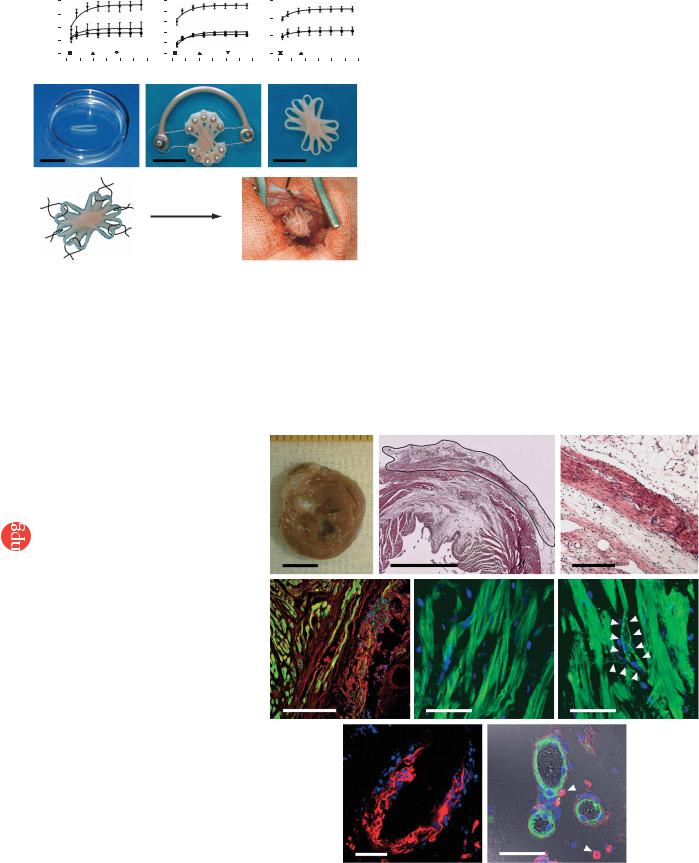
© 2006 Nature Publishing Group http://www.nature.com/naturemedicine
a |
0.8 |
|
* |
b |
|
* |
c |
|
|
|
||
|
|
|
|
3 |
|
* |
||||||
|
|
|
|
|||||||||
(mN) |
|
|
2 |
|
|
|
|
|
||||
0.4 |
|
|
|
|
2 |
|
|
|
||||
|
|
|
|
|
|
|
|
|
|
|||
|
|
|
|
|
|
|
|
|
|
|
|
|
TT |
|
|
1 |
|
|
|
1 |
|
|
|
||
|
|
|
|
|
|
|
|
|
|
|
||
|
|
|
|
|
|
|
|
|
|
|
|
|
|
0 |
|
|
21% 30% 40% O2 0 |
|
|
Sta. Pha. Aux. |
0 |
|
|
Ctr. Insulin |
|
|
|
|
|
|||||||||
|
|
|
|
|
|
|
|
|
|
|
|
|
0 |
1 |
2 |
3 |
0 |
1 |
2 |
3 |
0 |
1 |
2 |
3 |
|
Calcium (mmol/l) |
|
|
Calcium (mmol/l) |
|
|
Calcium (mmol/l) |
|
|||
d |
|
|
e |
|
|
|
|
f |
|
|
|
g
engraftment were evaluated by three independent means including echocardiography, magnetic resonance imaging (MRI) and left heart catheterization; (iv) acquisition of echocardiography, MRI and catheterization data and their evaluation were carried out by independent investigators blinded to the study protocol.
RESULTS |
|
|
Construction of improved EHT grafts |
|
|
Three modifications of the original reconsti- |
|
|
tution protocol improved EHT: (i) culture |
|
|
under elevated ambient oxygen (Fig. 1a), (ii) |
a |
|
culture under auxotonic load (that is, EHT |
||
|
||
Figure 2 EHT morphology 4 weeks after |
|
|
engraftment. (a) Mid-ventricular cross-section |
|
|
28 d after implantation showed that engrafted |
|
|
EHTs remained pale but firmly attached to the |
|
|
infarct scar. (b,c) H&E staining of paraffin |
|
|
sections through the infarcted left ventricular free |
|
|
wall 4 weeks after EHT engraftment showed the |
d |
|
formation of thick cardiac muscle on top of the |
||
infarct scar (b; EHT encircled). High-power |
|
|
magnification of the infarct area showed that |
|
|
engrafted EHTs formed compact and oriented |
|
|
heart muscle (c) spanning the transmural infarct. |
|
|
(d) Engrafted EHTs could be identified by DAPI- |
|
|
labeled nuclei (blue; actin, green; background, |
|
|
red). (e) Laser scanning microscopy showed the |
|
|
highly differentiated sarcomeric organization of |
|
|
engrafted cardiomyocytes (actin, green; nuclei, |
|
|
blue). (f–h) EHT grafts were vascularized in vivo |
|
|
(f; actin, green; nuclei, blue; arrows indicate a |
g |
|
putative vessel in a reconstituted confocal |
||
|
||
image). Newly formed vessels contained DAPI+ |
|
|
endothelial (g; lectin, red; nuclei, blue) or smooth |
|
|
muscle cells (h; actin, green; nuclei, blue). |
|
|
Macrophages (ED2, red; highlighted by arrows in |
|
|
h) with blue nuclei were found in close proximity |
|
|
to newly formed vessels. Asterisks indicate |
|
|
erythrocytes visualized by differential interference |
|
|
contrast imaging (h). Scale bar in a,b, 5 mm; |
|
|
in c,d, 500 mm; in e–h, 50 mm. |
|
T E C H N I C A L R E P O R T S
Figure 1 Construction of optimized EHTs. Effects of oxygen, load and insulin were analyzed by isometric contraction experiments in EHT rings. TT, twitch tension. Direct comparison of calcium response curves showed that EHTs benefited from oxygen supplementation (O2, n ¼ 11 at 21%; n ¼ 5 at 30%; n ¼ 10 at 40%; a), auxotonic (Aux.; n ¼ 8) in contrast to
static (Sta.; n ¼ 8) or phasic (Pha.; n ¼ 8) load (b), and addition of insulin (10 mg/ml; n ¼ 4) when compared to untreated controls (n ¼ 3; c). Stacking five single EHTs (d) on a custom-made device facilitated EHT fusion and allowed contractions under auxotonic load (e), resulting in synchronously contracting multiloop EHTs (f and Supplementary Video 1 online) ready for in vivo engraftment. (g) Six single-knot sutures served to fix multiloop EHTs on the recipients’ hearts. * P o 0.05 versus 21% O2, static load and culture in the absence of insulin (Ctr.), respectively, by repeated ANOVA. Scale bars, 10 mm.
contract against load-adjusted coils; Fig. 1b) and (iii) supplementation of the culture medium with insulin (Fig. 1c). The increase in force was paralleled by an increase in total and muscle-specific calsequestrin (CSQ2) protein (Supplementary Fig. 1 online). DNA content was not affected, indicating either an increased fraction of cardiomyocytes without a relevant change in total EHT cell number or advanced development of the sarcoplasmic reticulum in cardiomyocytes. To increase graft size and facilitate implantation, we stacked five circular EHTs (Fig. 1d) crosswise on a custom-made holding device after 7 d in culture (Fig. 1e). Under these conditions, circular EHTs fused and formed synchronously contracting multiloop EHTs (diameter, B15 mm; thickness, 1–4 mm; Fig. 1f and Supplementary Video 1 online). Multiloop EHTs consisted of longitudinally oriented, thoroughly interconnected muscle strands (Supplementary Fig. 2 online) and contracted spontaneously at 1–2 Hz.
b |
c |
e |
f |
h
*
*
*
NATURE MEDICINE VOLUME 12 [ NUMBER 4 [ APRIL 2006 |
4 5 3 |
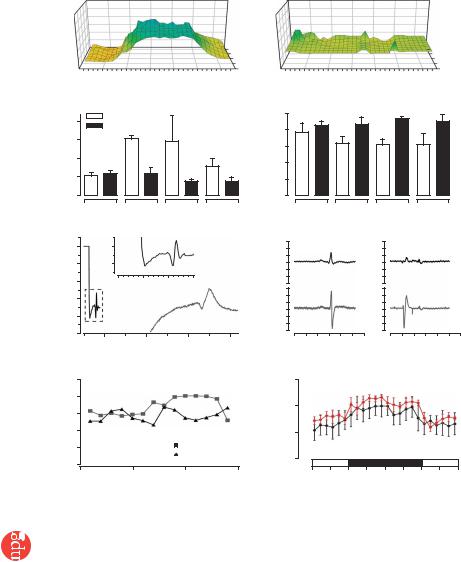
|
T E C H N I C A L R E P O R T S |
|
|
|
|
|
|
|
|
|
|
|||||||
|
a |
|
|
Right |
Anterior Lateral Posterior |
b |
|
Right |
Anterior Lateral Posterior |
|
|
|
||||||
|
|
|
|
|
|
|
|
|
|
|
|
|
|
|
|
|
||
|
(ms) |
30 |
|
|
|
|
|
|
30 |
|
|
|
|
|
|
|
|
|
|
time |
20 |
|
|
|
|
|
|
20 |
|
|
|
|
|
|
y |
|
|
|
Activation |
|
|
|
|
|
|
|
|
|
|
|
|
|
|
|
|
|
|
|
|
|
|
|
|
|
|
|
|
|
|
|
|
8 |
- |
|
|
|
10 |
|
|
|
|
|
|
10 |
|
|
|
|
|
axis |
||||
|
|
|
|
|
|
|
|
|
|
|
|
|
||||||
|
|
|
|
|
|
|
|
|
|
|
|
|
|
|
4Electrode |
|||
|
0 |
|
|
|
|
|
|
|
0 |
|
|
|
|
|
|
(#) |
||
|
|
8 |
16 |
|
24 |
32 |
|
|
|
|
|
|
|
1 |
|
|||
|
1 |
|
|
|
1 |
8 |
|
16 |
24 |
32 |
|
|
||||||
|
|
|
|
|
|
|
|
|||||||||||
|
c |
|
|
Electrode x-axis (#) |
|
d |
|
Electrode x-axis (#) |
|
|
|
|
||||||
|
|
|
|
|
|
|
|
5 |
|
|
|
* |
|
|
|
|
||
|
|
20 |
|
Sham; n = 5 |
|
|
|
|
|
|
|
|
|
|
|
|||
|
|
|
EHT; n = 8 |
|
|
|
|
4 |
|
|
|
|
|
|
|
|
||
|
|
|
|
|
|
|
|
|
|
|
|
|
|
|
|
|||
|
|
|
|
|
|
|
|
|
|
|
|
|
|
|
|
|
|
|
|
|
|
|
|
|
|
|
|
|
3 |
|
|
|
|
|
|
|
|
|
|
10 |
|
|
* |
|
|
|
|
2 |
|
|
|
|
|
|
|
|
|
|
|
|
|
|
* |
|
|
|
|
|
|
|
|
|
|
||
|
|
|
|
|
|
|
|
|
1 |
|
|
|
|
|
|
|
|
|
|
|
|
|
|
|
|
|
|
|
|
|
|
|
|
|
|
|
|
|
|
0 |
|
|
|
|
|
|
|
0 |
|
|
|
|
|
|
|
|
|
|
|
Right |
Anterior |
Lateral |
Posterior |
|
|
Right |
Anterior |
Lateral |
Posterior |
|
|||||
|
e |
|
|
Myocardial segments |
|
f |
|
|
Myocardial segments |
|
|
|
||||||
|
|
|
EHT |
|
|
|
|
pH 7.4 |
|
|
pH 6.7 |
|
|
|
||||
http://www.nature.com/naturemedicine |
mV activationTotal(ms)time |
4 |
|
|
|
|
mV amplitudemV QRS(mV) |
|
|
|
|
|
|
|
|
|
||
|
1 |
|
|
|
|
2 |
|
|
|
EHT |
|
|
|
|
||||
|
mV |
|
|
|
|
|
EHT |
|
|
|
|
|
|
|||||
3 |
0 |
|
|
|
|
0 |
|
|
|
|
|
|
|
|
||||
2 |
130 |
135 |
140 |
145 |
|
–2 |
|
|
|
|
|
|
|
|
||||
|
Myocardium |
|
Myocardium |
|
|
|||||||||||||
|
|
ms |
|
|
|
|
|
|
||||||||||
1 |
|
|
|
|
|
2 |
|
|
|
|
|
|
|
|
||||
|
|
|
|
|
|
|
|
|
|
|
|
|
|
|||||
0 |
|
|
|
|
|
0 |
|
|
|
|
|
|
|
|
||||
|
|
|
Remote |
–2 |
|
|
|
|
|
|
|
|
||||||
Group |
|
|
|
|
|
|
|
|
|
|
|
|
|
|
||||
|
|
|
|
|
|
myocardium |
|
|
|
|
|
|
|
|
|
|||
|
–1 |
|
|
|
|
|
|
|
|
|
|
|
|
|
|
|||
|
150 |
200 |
|
250 |
300 |
|
0 |
200 |
400 |
600 |
0 |
200 |
400 |
600 |
||||
|
|
|
|
|||||||||||||||
|
|
Time after point stimulation (ms) |
|
|
ms |
|
|
ms |
|
|
||||||||
g |
|
|
|
|
|
|
|
h |
|
|
|
|
|
|
|
|
|
|
Nature Publishing |
105 |
|
|
|
|
|
|
500 |
|
|
|
|
|
|
|
|
||
Arrhythmic events |
|
|
|
|
|
|
Heat rate (b.p.m.) |
|
|
|
|
Sham |
|
|||||
104 |
|
|
|
|
|
|
|
|
|
|
|
EHT |
|
|||||
|
|
|
|
|
|
|
|
|
|
|
|
|
|
|
||||
103 |
|
|
|
|
|
|
400 |
|
|
|
|
|
|
|
|
|||
|
|
|
|
|
|
|
|
|
|
|
|
|
|
|
||||
102 |
|
|
|
|
|
|
300 |
|
|
|
|
|
|
|
|
|||
101 |
|
|
|
|
Sham |
|
|
|
|
|
Dark |
|
|
|
|
|||
100 |
|
|
|
|
EHT |
|
200 |
|
|
|
|
|
|
|
||||
|
|
|
|
|
|
|
|
|
|
|
|
|
|
|||||
|
|
|
|
|
|
|
|
|
|
|
|
|
|
|
||||
2006 |
|
0 |
|
|
10 |
|
20 |
30 |
|
|
13:00 |
19:00 |
1:00 |
7:00 |
|
13:00 |
||
|
|
|
Day of telemetric recording |
|
|
|
|
|
Time |
|
|
|
|
|||||
|
|
|
|
|
|
|
|
|
|
|
|
|
|
|
|
|
|
|
© |
|
|
|
|
|
|
|
|
|
|
|
|
|
|
|
|
|
|
Figure 3 Electrical integration of EHTs in vivo. Representative plots of epicardial activation times in sham-operated (a) and EHT-engrafted (b) hearts. Total activation time (c) and QRS-complex voltage (d) in right, anterior, lateral and posterior segments of the investigated hearts.
(e) Point stimulation of an implanted EHT with simultaneous recording of the propagated potential in the EHT and in remote myocardium showed retrograde coupling. EHT could be uncoupled after acidification of the hearts (f). Telemetric ECG recordings showed a similar frequency of arrhythmias in sham-operated and EHT-grafted groups (n ¼ 5 per group; g).
(h) Circadian rhythm in sham-operated and EHT groups. *P o 0.05 versus sham-operated rats by ANOVA with Mann-Whitney U-test.
fluorescence of the DAPI-labeled nuclei (Fig. 2d and Supplementary Fig. 4 online). EHT-derived cardiomyocytes developed a differentiated phenotype, including in registry organization of sarcomeres (Fig. 2e). We did not observe DAPI+ cardiomyocytes in the host myocardium or DAPI– cardiomyocytes within the EHT graft. Notably, blood vessels in the EHT grafts also contained DAPI+ cells (Fig. 2f–h) including endothelial cells (Fig. 2g) and smooth muscle cells (Fig. 2h), suggesting that they were of donor origin. Identification of erythrocytes in these vessels indicated that they were connected to the recipient’s vasculature (Fig. 2h). Whether DAPI+ macrophages detected in close vicinity to these vessels (Fig. 2h) have a role in de novo vascularization remains to be investigated.
EHT grafting on infarcted hearts
We implanted multiloop EHTs into male Wistar rats with large myocardial infarcts. Sham-operated (stitches only) rats served as negative controls, and implantation of either formaldehyde-fixed EHTs or noncardiomyocyte grafts (NCMGs) served as tests for effects of scar stabilization and supply of cardiac nonmyocyte–derived paracrine factors (Supplementary Table 1 online). We performed all interventions 14 d after left descending coronary artery (LAD) ligation in rats with compromised myocardial function (fractional area shortening (FAS) o40% by echocardiography; Fig. 1g and Supplementary Note and Supplementary Fig. 3 online).
Structural integration and integrity of EHT in vivo
Four weeks after grafting, EHTs could be identified by their distinctive appearance and location (Fig. 2a). The pale color indicated that EHTs retained a high connective-tissue content. Hematoxylin and eosin (H&E)-stained paraffin sections, however, showed compact and welldifferentiated EHT-derived heart muscle (diameter, 443 ± 32 mm; n ¼ 5) covering the infarcted myocardium (Fig. 2b,c). Noncontractile grafts did not show any cardiac muscle structures. To further evaluate whether cardiomyocytes within the reconstituted myocardium were of donor origin, we labeled EHTs with DAPI (1 mg/ml) before implantation (Supplementary Fig. 4 online) and searched for DAPI+ cells 4 weeks after EHT implantation (n ¼ 10). Engrafted EHTs could be clearly distinguished from the recipient’s myocardium by the blue
Electrical coupling of EHT grafts to host myocardium
We assessed electrical coupling of EHTs to the host myocardium 4 weeks after engraftment by high-resolution epicardial mapping28 (Fig. 3). Sham-operated rats (n ¼ 5) showed the expected delay of impulse propagation in infarcted anterior and lateral segments (Fig. 3a,c). In contrast, epicardial activation was normal in EHTgrafted hearts (n ¼ 8), indicating undelayed anterograde coupling of EHTs to the host myocardium (Fig. 3b,c). QRS amplitude in lateral segments was higher in the EHT group than in the sham-operated group, providing electrophysiological evidence for myocardial reconstitution (Fig. 3d). Hearts that received noncontractile grafts (n ¼ 4) showed similar conduction deficits as shamoperated hearts and showed no active responses in most areas of the grafts.
Pacing of implanted EHTs resulted in propagated electrical responses in remote myocardium, indicating retrograde coupling (n ¼ 2; Fig. 3e). Pacing through noncontractile grafts (formalde- hyde-fixed EHTs, n ¼ 3; NCMGs, n ¼ 2) did not provoke local or propagated potentials. Acidification of the perfusion buffer to pH 6.7 led to uncoupling of EHTs from the native myocardium, providing additional proof for direct but subnormal graft-host coupling (Fig. 3f). Maximum conduction velocity in engrafted EHTs was 0.55 ± 0.16 m/s longitudinally (VL) and 0.16 ± 0.07 m/s transversally (VT; n ¼ 3), comparable to conduction velocity in noninfarcted myocardium (VL, 0.69 ± 0.06 m/s; VT, 0.19 ± 0.05 m/s; n ¼ 3).
4 5 4 |
VOLUME 12 [ NUMBER 4 [ APRIL 2006 NATURE MEDICINE |
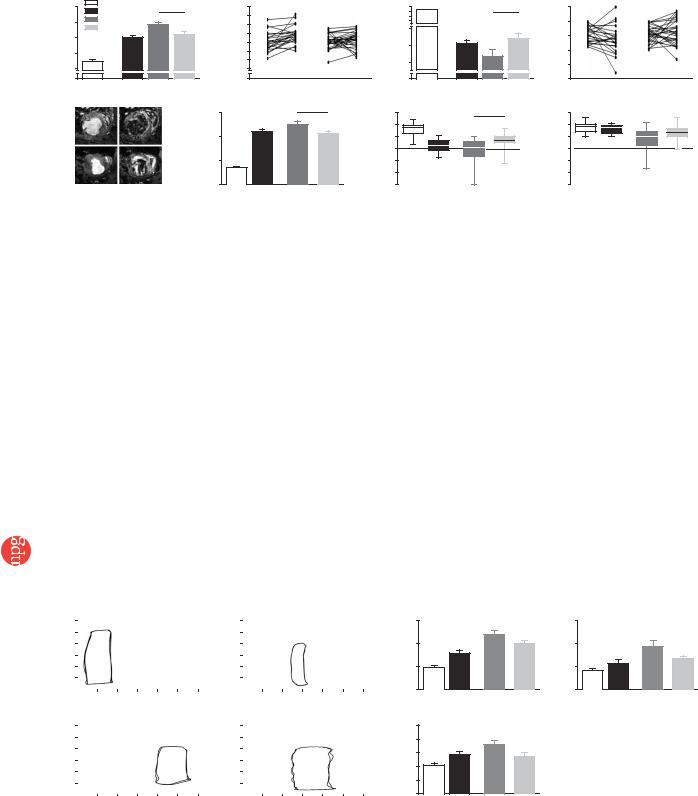
|
|
|
|
|
|
|
|
|
|
|
|
|
|
T E C H N I C A L R E P O R T S |
||||||
|
a 11 |
Healthy |
|
P < 0.05 |
b |
|
Sham |
EHT |
|
c |
70 |
P < 0.05 |
d 50 |
Sham |
|
EHT |
||||
|
MI baseline |
|
|
|
||||||||||||||||
|
|
|
|
|
12 |
|
|
|
|
|
50 |
|
|
|
|
|
|
|
||
|
LVEDD (mm) |
10 |
Sham |
|
|
LVEDD (mm) |
|
|
|
|
Percent FAS |
|
Percent FAS |
40 |
|
|
|
|
||
|
|
|
|
|
|
|
|
|
|
|
|
|
|
|||||||
|
EHT |
|
|
|
|
|
|
|
35 |
|
|
|
|
|
||||||
|
|
|
|
10 |
|
|
|
|
|
30 |
|
|
|
|
||||||
|
9 |
|
|
|
|
|
|
|
30 |
|
|
|
|
|
||||||
|
8 |
|
|
|
8 |
|
|
|
|
|
20 |
|
|
|
|
|||||
|
|
|
|
|
|
|
|
|
|
|
|
|
|
|||||||
|
7 |
|
|
|
6 |
P < 0.001 |
P = 0.4 |
25 |
|
10 |
P = 0.07 |
P = 0.6 |
||||||||
|
|
|
|
|
|
|
|
|
|
|
||||||||||
|
|
0 |
|
|
|
|
0 |
|
0 |
|
|
0 |
||||||||
|
|
|
|
|
|
Pre |
Post |
Pre |
Post |
|
|
|
Pre |
Post |
Pre |
Post |
||||
|
|
|
|
|
|
|
|
|
|
|
|
|
||||||||
|
e |
|
Systole |
Diastole |
f |
|
|
|
P = 0.07 |
g |
|
|
P < 0.01 |
h |
|
|
|
|
|
|
|
|
|
|
|
l) |
1,200 |
|
|
|
75 |
|
|
75 |
|
|
|
|
|||
|
|
EHT Sham |
|
|
|
|
|
|
|
|
|
|
|
|
|
|||||
http://www.nature.com/naturemedicine |
|
|
|
Max. LV volume ( |
|
|
|
|
|
Percent AWThF |
50 |
|
|
Percent PWThF |
50 |
|
|
|
|
|
|
|
|
800 |
|
|
|
|
25 |
|
|
25 |
|
|
|
|
|||||
|
|
|
|
|
|
|
|
0 |
|
|
0 |
|
|
|
|
|||||
|
|
|
400 |
|
|
|
|
–25 |
|
|
–25 |
|
|
|
|
|||||
|
|
|
|
|
|
|
|
–50 |
|
|
–50 |
|
|
|
|
|||||
|
|
|
0 |
|
|
|
|
–75 |
|
|
–75 |
|
|
|
|
|||||
|
|
|
|
|
|
|
|
|
|
|
|
|
|
|
|
|
||||
Figure 4 Changes in left ventricular function after EHT implantation. Left ventricular end-diastolic diameter (LVEDD; a,b) and FAS (c,d) determined by echo- |
||||||||||||||||||||
cardiography. ‘Healthy’ indicates age-matched untreated controls (n ¼ 29). ‘MI baseline’ indicates pooled values (n ¼ 53) measured 14 d after myocardial |
||||||||||||||||||||
infarction in rats that underwent sham (n ¼ 24) or EHT (n ¼ 29) surgery. These rats were reevaluated after an additional 28 d. Trajectories show the change |
||||||||||||||||||||
of LVEDD (b) and FAS (d) for each rat. Pre, values at MI baseline; Post, values 28 d after sham or EHT surgery. MRI was performed subsequently (e–h) with |
||||||||||||||||||||
five exceptions due to technical reasons. Maximal and minimal volumes of the left ventricle were calculated from 4D CINE mode MRI images in EHT-grafted |
||||||||||||||||||||
(n ¼ 24) and sham-operated (n ¼ 19) rats (e). Analyses of systolic thickening in anterior (AWThF; g) and posterior (PWThF; h) segments of the ventricular |
||||||||||||||||||||
wall served to assess local contractility. MRI data from healthy rats (n ¼ 29) and 2 weeks after infarction (n ¼ 8) were recorded in an independent series of |
||||||||||||||||||||
experiments and are shown for comparison. LV, left ventricular. Statistical evaluations were performed by unpaired (a,b,f–h) and paired (b,d) Student t-tests. |
||||||||||||||||||||
|
|
|
|
|
|
|
|
|
|
|
|
|
|
|
|
|
|
|
|
© 2006 Nature Publishing Group
Anisotropy (VL/VT) was similar in EHT-grafted and noninfarcted myocardium (4.15 ± 0.8 versus 4.07 ± 0.91).
Electrocardiographic (ECG) telemetry in sham-operated and EHTgrafted rats (n ¼ 5 each) showed that EHT engraftment did not increase total number, frequency or severity of arrhythmias (Fig. 3g and Supplementary Fig. 5 online) and that circadian rhythm was restored 4 d after EHT or sham surgery (Fig. 3h).
Functional consequences of EHT grafting
We performed echocardiographic examinations 14 d after LAD ligation in all surviving rats (n ¼ 172). Seventy rats had a FAS below 40%, survived the complete study and were subsequently subjected to echocardiography (70 of 70), MRI (59 of 70) and catheterization (50 of 70) 28 d after EHT or noncontractile graft implantation and sham surgery (Supplementary Tables 1–4 online). In contrast to echocardiography which had a longitudinal study design, we refrained
from repeated MRI and catheterization because of the high mortality during MRI (1–2 h under anesthesia) and the availability of only one defined access to the left ventricle through the right carotid artery (catheterization).
Evaluation 14 d after infarction (Figs. 4 and 5 and Supplementary Tables 2–4 online) showed enlargement of left ventricular dimension and volume (Figs. 4a,f and 5b), reduced FAS (Fig. 4c), decreased anterior wall thickening fraction (AWThF; Fig. 4g) without notable effects on the posterior wall thickening fraction (PWThF; Fig. 4h), increased left ventricular end-diastolic pressure (LVEDP; Fig. 5c) and tau (index of relaxation; Fig. 5d) and rightward-shifted pressurevolume loops (Fig. 5a).
Twenty-eight days later, echocardiography, MRI and catheterization data collectively indicated further deterioration in sham-operated rats (Figs. 4 and 5 and Supplementary Tables 2–4 online). In particular, left ventricular end-diastolic dimension (LVEDD) increased
a
Pressure (mmHg) Pressure (mmHg)
120 |
|
|
|
|
|
Healthy control |
||
|
|
|
|
|||||
100 |
|
|
|
|
|
|
|
|
80 |
|
|
|
|
|
|
|
|
60 |
|
|
|
|
|
|
|
|
40 |
|
|
|
|
|
|
|
|
20 |
|
|
|
|
|
|
|
|
0 |
|
|
|
|
|
|
|
|
0 |
200 |
400 |
600 |
800 |
1,000 1,200 |
|||
|
|
|
|
Volume ( l) |
|
|
||
120 |
|
|
|
|
6 weeks after MI |
|||
|
|
|
|
|||||
100 |
|
|
|
|
Sham |
|
|
|
80 |
|
|
|
|
|
|
|
|
60 |
|
|
|
|
|
|
|
|
40 |
|
|
|
|
|
|
|
|
20 |
|
|
|
|
|
|
|
|
0 |
|
|
|
|
|
|
|
|
0 |
200 |
400 |
600 |
800 |
1,000 1,200 |
Volume ( l)
Pressure (mmHg) Pressure (mmHg)
120 |
|
|
|
|
2 weeks after MI |
|||
|
|
|
||||||
100 |
|
|
|
|
|
|
|
|
80 |
|
|
|
|
|
|
|
|
60 |
|
|
|
|
|
|
|
|
40 |
|
|
|
|
|
|
|
|
20 |
|
|
|
|
|
|
|
|
0 |
|
|
|
|
|
|
|
|
0 |
200 |
400 |
600 |
800 |
1,000 1,200 |
|||
|
|
|
|
Volume ( l) |
|
|
||
120 |
|
|
|
|
6 weeks after MI |
|||
|
|
|
|
|||||
100 |
|
|
|
|
EHT |
|
|
|
80 |
|
|
|
|
|
|
|
|
60 |
|
|
|
|
|
|
|
|
40 |
|
|
|
|
|
|
|
|
20 |
|
|
|
|
|
|
|
|
0 |
|
|
|
|
|
|
|
|
0 |
200 |
400 |
600 |
800 |
1,000 1,200 |
Volume ( l)
b 1,200 LV-cath |
P = 0.07 |
c 30 |
800 |
LVEDP(mmHg) |
20 |
400 |
10 |
|
LVEDVl)( |
|
|
0 |
|
0 |
d |
25 |
P < 0.05 |
|
||
(ms) |
20 |
|
15 |
|
|
|
|
|
tau |
10 |
|
5 |
|
|
|
|
|
|
0 |
|
P < 0.05
Healthy
MI baseline
Sham
EHT
Figure 5 Influence of EHT grafting on left ventricular hemodynamics. (a) Representative pressure-volume loops and detailed analyses of left ventricular end-diastolic volume (LVEDV, b), pressure (LVEDP, c) and relaxation (tau, d) in EHT-grafted (n ¼ 7) and sham-operated rats (n ¼ 17). Left ventricular catheterization (LV-cath) data from healthy rats (n ¼ 6) and 2 weeks after infarction (n ¼ 9) were recorded in an independent series of experiments and are shown for comparison. Statistical evaluations were performed by unpaired Student t-tests. MI, myocardial infarction.
NATURE MEDICINE VOLUME 12 [ NUMBER 4 [ APRIL 2006 |
4 5 5 |

T E C H N I C A L R E P O R T S
© 2006 Nature Publishing Group http://www.nature.com/naturemedicine
(Fig. 4a,b) and FAS decreased (Fig. 4c,d). Moreover, LVEDP and tau increased markedly (Fig. 5c,d). In contrast, LVEDD and FAS remained unchanged in the EHT-grafted group; that is, LVEDD and FAS did not differ from baseline values 2 weeks after LAD ligation (Fig. 4a–d). In fact, retrospective stratification according to FAS (20–30% versus 30–40% at baseline) showed that FAS significantly (P ¼ 0.01) increased after EHT implantation in rats with lower baseline FAS (Supplementary Fig. 3 and Supplementary Tables 2–4 online).
MRI (Fig. 4e–h) supported the echocardiography data, showing smaller left ventricular volumes in EHT-grafted rats when compared to sham-operated rats (Fig. 4f). Moreover, MRI-recorded AWThF was markedly better in EHT-grafted rats when compared to sham-oper- ated rats (Fig. 4g). PWThF was not affected (Fig. 5h). In the group of rats that received noncontractile grafts (Supplementary Tables 2–4 online), AWThF was similar to sham-operated and myocardial infarction baseline values.
Pressure-volume loop analyses (Fig. 5a–d) supported these data, showing that left ventricular dilation was less (Fig. 5b) and LVEDP as well as tau were markedly decreased in EHT-grafted rats compared to sham-operated rats (Fig. 5c,d). Notably, EHT grafting resulted in a better catheterization-determined ejection fraction only in rats with a low baseline FAS (Supplementary Fig. 3 online).
DISCUSSION
We evaluated the hypothesis that myocardial function after infarction can be improved by EHT grafts. Our data show that EHTs integrate and electrically couple to host myocardium and exert beneficial effects on systolic and diastolic left ventricular function. Although we neither observed nor expected complete reversal of myocardial dysfunction after EHT engraftment, we believe that our study can serve as a proof of principle for a tissue engineering approach in repair of cardiac muscle.
A prerequisite for successful cardiac repair is that grafted cells electrically and mechanically couple to native myocardium and do not elicit arrhythmias29. Here, we observed normalization of epicardial impulse propagation after EHT engraftment and observed no evidence for arrhythmogenicity. This normalization occurs despite spontaneous electrical activity of EHTs, suggesting that with the establishment of electrical coupling, EHTs are likely to be overpaced by the recipient heart, beating at B5 Hz (EHTs, 1–2 Hz) and may then chronically adapt by electrical conditioning. Another reason for the apparent lack of EHT-induced arrhythmias despite electrical coupling may be that electrical contacts between EHT and recipient myocardium were subnormal, resulting in a low propensity of retrograde myocardial activation by EHT. This notion is supported by the finding that EHTs could be uncoupled from recipient myocardium by pH lowering. Proarrhythmic risks of EHT implantation, however, are likely to be of more relevance in larger, low-heart-rate species including humans and will need further investigation.
The observation that EHTs structurally and electrically integrate into host myocardium is not trivial given the fact that EHTs are not homogeneous heart muscles but organoids consisting of muscle strands, primitive capillaries, fibroblasts, smooth muscle cells and macrophages in a collagen matrix23. One may ask how cardiomyocytes and other structures in EHTs contact host myocardium. A possible explanation is that the implantation procedures injure EHTs and host myocardium and thereby destroy physiological barriers (epicardium of native hearts and nonmyocyte surface lining of EHTs). In addition, spontaneous fusion of single EHTs to multiloop configurations indicates a marked remodeling capacity of EHTs. The observation that blood vessels inside implanted EHTs were DAPI+ is intriguing and
implies that they are, at least in part, derived from EHTs. This may seem unexpected, but we have previously observed primitive capillaries in EHTs in vitro23. We have also documented that hostderived blood vessels start to invade EHTs early after implantation27. Thus, the most probable explanation is that host blood vessels contact primitive vascular structures in EHTs or that donor cells may be recruited during de novo vascularization. This may contribute to the survival and formation of thick muscle structures after implantation.
We took care to study functional consequences of EHT implantation under well-controlled conditions. These conditions include the well-validated rat LAD-ligation model, large groups of rats with predefined inclusion criteria, sham-operated and noncontractile graft controls, determination of heart function by three different high-end technologies and data evaluation by indepen-
dent investigators |
blinded to the experimental conditions. Only |
70 out of 343 |
(23%) experimental rats completed the study. |
This was mainly a consequence of high mortality after myocardial infarction (172 of 343 survived; 50%) as well as variability of infarct size and its functional consequences (80 of 172 had a FAS of 440%). We made the following observations: EHT-grafted hearts were smaller, had lower LVEDP and tau and better FAS and AWThF; that is, they showed better diastolic and systolic function. Our longitudinal echocardiography studies showed that part of this difference resulted from preservation of the myocardial infarction baseline state. We observed that rats with the worst myocardial baseline function (FAS, 20–30%), however, showed a substantial improvement after EHT engraftment.
The fact that we did not observe a similar effect in rats with noncontractile grafts suggests that the improvement of regional systolic function resulted from cardiomyocyte engraftment and not merely from scar stabilization or paracrine effects. Noncontractile grafts seemed to exert some beneficial effects on ventricular dimensions and ejection fraction, possibly resulting from ventricle-stabilizing effects. The absence of DAPI+ cardiomyocytes in recipient myocardium or DAPI– cardiomyocytes in engrafted DAPI-labeled EHTs argue against a substantial contribution of endogenous or exogenous cardiac progenitors.
Yet is improvement of cardiac function a realistic consequence of EHT implantation? After implantation, we observed the formation of new compact heart muscle with a thickness of 443 ± 32 mm. This thickness is close to the size of a rat papillary muscle and could indeed account for a relevant improvement of wall motion. Future experiments will have to evaluate whether further EHT conditioning in vitro or optimized implantation techniques will improve the functional effect of EHT grafting.
Several important questions remain in terms of a potential clinical
application. The most obvious relates to a suitable cell source. Autologous stem cells with a cardiogeneic potential13,14,19,20,30 would
be ideal, and we are currently evaluating whether they can be used for generating EHTs. The EHT culture format may in fact provide a milieu that supports cardiac differentiation23. Preliminary experiments with human embryonic stem cells support this notion, offering a perspective for the generation of human EHTs (W.-H.Z., I. Kehat & L. Gepstein, unpublished data). A second problem is the immunogenicity of EHTs in their present form27, and we are currently trying to establish serumand Matrigel-free culture conditions. A third caveat pertains to graft size, and it is not clear whether the functional outcome will improve with the number of EHTs implanted. Despite these and other open questions, our study provides a realistic therapeutic perspective for cardiac tissue engineering.
4 5 6 |
VOLUME 12 [ NUMBER 4 [ APRIL 2006 NATURE MEDICINE |

T E C H N I C A L R E P O R T S
© 2006 Nature Publishing Group http://www.nature.com/naturemedicine
METHODS
Animal care and experimental protocol approval. We maintained experimental rats in accordance with the guiding principles of the American Physiological Society. The study was approved by local authorities (Regierung von Mittelfranken: 621.2531.31-2/00 and -17/01; BWG of the Freie und Hansestadt Hamburg: #54/04).
EHT construction. We constructed EHTs as previously described27. Briefly, we prepared EHT rings (reconstitution volume, 0.9 ml) by mixing isolated heart cells from neonatal rats (2.5 106 cells/EHT) with collagen type I from rat tails (0.8 mg/EHT), Engelbreth-Holm-Swarm tumor exudate (10% vol/vol; tebu) and concentrated serum-containing culture medium (2 DMEM, 20% horse serum, 4% chick embryo extract, 200 U/ml penicillin and 200 mg/ml streptomycin); we neutralized pH with 0.1 N NaOH. We transferred EHTs after 7 d onto custom-made stretch devices to facilitate static (110% of slack length), phasic (from 100 to 110% of slack length at 2 Hz) or auxotonic (culture on coils at 110% of slack length adjusted to deflect 1 mm at 1–1.5 mN) loading. We analyzed contractile parameters by isometric contraction experiments of single-loop EHTs as previously described27. We generated multiloop EHTs by stacking five single EHTs. To control for indirect paracrine or matrix-mediated effects, we used formaldehyde-fixed grafts and NCMGs.
Infarct model and EHT grafting. We generated myocardial infarctions in ventilated, isoflurane (2%)-anesthetized male Wistar rats (324 ± 2 g; n ¼ 343) by permanent LAD ligation (5-0, Prolene, Ethicon). We implanted EHTs and noncontractile grafts 14 d after infarct induction (inclusion criterion: FAS o40% by echocardiography; Supplementary Note and Supplementary Fig. 3 online) with six single-knot sutures (Fig. 1g; 5-0, Prolene, Ethicon). We placed the sutures in healthy myocardium adjacent to the visible infarct scars to arrange the center of the EHTs just above the latter. In the sham-operated group, sutures were placed as if EHTs were implanted. All rats received immunosuppressants as previously described (mg/kg body weight/d: azathioprine, 2; cyclosporin A, 5; methylprednisolone, 5)27.
Histology. We fixed hearts in neutral buffered 4% formaldehyde–1% methanol, pH 7.4, and subjected them to histological analysis as previously described27. Briefly, we stained paraffin-embedded sections (4 mm) with H&E. We stained cryosections (10 mm) with phalloidin–Alexa 488 (3.3 U/ml; Molecular Probes) to label F-actin, Bandeiraea simplicifolia lectin-TRITC (10 mg/ml; Sigma) to label endothelial cells and antibodies directed against ED2 (CD163 cell-surface glycoprotein, 1:100; Serotec) to label macrophages with appropriate secondary antibodies.
Epicardial mapping. We performed epicardial multielectrode mapping as previously described28 (Supplementary Methods online).
Ambulatory ECG telemetry. We inserted telemetry devices (PhysioTel CA-F40, DataSciences) into the abdominal cavity during sham operation (n ¼ 5) or EHT (n ¼ 5) operation to record electrocardiograms continuously for 2 (sham/EHT, n ¼ 2 per group) to 4 (n ¼ 3 per group) weeks. Data analysis was performed semiautomatically (ECG-auto, v.1.5.12.38, EMKA Technologies) using rat-specific waveform libraries (Supplementary Methods online).
Echocardiography. We performed echocardiography in volatile isoflurane (2%) anesthesia as described previously with a HP Sonos 7500 System (Philips) equipped with a 15 MHz linear array transducer27 or a Vevo660 ultrasound biomicroscopy system equipped with a 37.5 MHz scan head (RMV 710; VisualSonics). Interand intraobserver variation of reported data was o10%.
Magnetic resonance imaging. We performed MRI in rats under volatile isoflurane (2%) anesthesia with a Bruker 4.7T Biospect System using a fast gradient echo sequence with 21 ms repetition time (TR), 5 ms echo time (TE) and a flip angle of 301. Recordings were ECG triggered and breath triggered. We acquired a total of six to eight sequential movie frames with 256 128 pixels at a pixel resolution of 200 400 mm. We obtained a longitudinal view for orientation purposes. Based on the latter, 20–30 cross-sections (short axis) from apex to base were imaged (4D cine mode). Subsequently, we subjected data sets to off-line analysis with manual segmentation of the heart contours.
Left ventricular catheterization. Pressure-volume loops were recorded under isoflurane (2%) anesthesia with a Millar 2 Fr catheter (model SPR-838) connected to Aria/PowerLab data-acquisition hardware (Millar/PowerLab) by an investigator blinded to the experimental conditions. We performed volume calibration by equating catheter-recorded minimal and maximal conductances with minimal and maximal MRI volumes, respectively. We analyzed all data off-line with PVAN 3.2 software (Millar).
Statistical analysis. Data are presented as mean ± s.e.m. or box plots indicating the median, 25th and 75th percentiles, and the range. We determined statistical differences using paired and unpaired two-tailed Student t-tests (in vivo data), repeated-measures ANOVA (in vitro contraction experiment) or MannWhitney U-test (mapping and telemetry). A P value of o0.05 was considered statistically significant.
Note: Supplementary information is available on the Nature Medicine website.
ACKNOWLEDGMENTS
This work is part of the doctoral thesis of I.M. at the Universities of ErlangenNuremberg and Hamburg. We acknowledge the technical assistance of S. John and F. Bussmann (University of Erlangen; MRI data evaluation) and T. Mu¨ller (University of Erlangen; construction of stretch devices). The help from H. Ru¨tten and D. Gehling (Aventis, Frankfurt) in echocardiography and pressure-volume loop recordings is appreciated. This study was supported by the German Research Foundation (Deutsche Forschungsgemeinschaft; Es 88/8-2 to T.E. and FOR 604/1-1 to T.E. and H.E.), the German Ministry for Education and Research (Bundesministerium fu¨r Bildung und Forschung 01GN 0124 and BMBF 01GN 0520 to T.E. and W.-H.Z.), the Deutsche Stiftung fu¨r Herzforschung (W.-H.Z.) and the Minerva foundation (W.-H.Z.).
COMPETING INTERESTS STATEMENT
The authors declare that they have no competing financial interests.
Published online at http://www.nature.com/naturemedicine/
Reprints and permissions information is available online at http://npg.nature.com/ reprintsandpermissions/
1.Murry, C.E., Field, L.J. & Menasche, P. Cell-based cardiac repair: reflections at the 10-year point. Circulation 112, 3174–3183 (2005).
2.Eschenhagen, T. & Zimmermann, W.H. Engineering myocardial tissue. Circ. Res. 97, 1220–1231 (2005).
3.Reinecke, H., Zhang, M., Bartosek, T. & Murry, C.E. Survival, integration, and differentiation of cardiomyocyte grafts: a study in normal and injured rat hearts. Circulation 100, 193–202 (1999).
4.Condorelli, G. et al. Cardiomyocytes induce endothelial cells to trans-differentiate into cardiac muscle: implications for myocardium regeneration. Proc. Natl. Acad. Sci. USA 98, 10733–10738 (2001).
5.Muller-Ehmsen, J. et al. Rebuilding a damaged heart: long-term survival of transplanted neonatal rat cardiomyocytes after myocardial infarction and effect on cardiac function. Circulation 105, 1720–1726 (2002).
6.Li, R.K. et al. Natural history of fetal rat cardiomyocytes transplanted into adult rat myocardial scar tissue. Circulation 96 Suppl., II-179–86; discussion 186–7 (1997).
7.Taylor, D.A. et al. Regenerating functional myocardium: improved performance after skeletal myoblast transplantation. Nat. Med. 4, 929–933 (1998).
8.Soonpaa, M.H., Koh, G.Y., Klug, M.G. & Field, L.J. Formation of nascent intercalated disks between grafted fetal cardiomyocytes and host myocardium. Science 264, 98–101 (1994).
9.Assmus, B. et al. Transplantation of Progenitor Cells and Regeneration Enhancement in Acute Myocardial Infarction (TOPCARE-AMI). Circulation 106, 3009–3017 (2002).
10.Orlic, D. et al. Bone marrow cells regenerate infarcted myocardium. Nature 410, 701–705 (2001).
11.Stamm, C. et al. Autologous bone-marrow stem-cell transplantation for myocardial regeneration. Lancet 361, 45–46 (2003).
12.Strauer, B.E. et al. Repair of infarcted myocardium by autologous intracoronary mononuclear bone marrow cell transplantation in humans. Circulation 106, 1913–1918 (2002).
13.Beltrami, A.P. et al. Adult cardiac stem cells are multipotent and support myocardial regeneration. Cell 114, 763–776 (2003).
14.Oh, H. et al. Cardiac progenitor cells from adult myocardium: homing, differentiation, and fusion after infarction. Proc. Natl. Acad. Sci. USA 100, 12313–12318 (2003).
15.Balsam, L.B. et al. Haematopoietic stem cells adopt mature haematopoietic fates in ischaemic myocardium. Nature 428, 668–673 (2004).
16.Murry, C.E. et al. Haematopoietic stem cells do not transdifferentiate into cardiac myocytes in myocardial infarcts. Nature 428, 664–668 (2004).
NATURE MEDICINE VOLUME 12 [ NUMBER 4 [ APRIL 2006 |
4 5 7 |

T E C H N I C A L R E P O R T S
© 2006 Nature Publishing Group http://www.nature.com/naturemedicine
17.Nygren, J.M. et al. Bone marrow-derived hematopoietic cells generate cardiomyocytes at a low frequency through cell fusion, but not transdifferentiation. Nat. Med. 10, 494–501 (2004).
18.Kehat, I. et al. Human embryonic stem cells can differentiate into myocytes with structural and functional properties of cardiomyocytes. J. Clin. Invest. 108, 407–414 (2001).
19.Laugwitz, K.L. et al. Postnatal isl1+ cardioblasts enter fully differentiated cardiomyocyte lineages. Nature 433, 647–653 (2005).
20.Messina, E. et al. Isolation and expansion of adult cardiac stem cells from human and murine heart. Circ. Res. 95, 911–921 (2004).
21.Eschenhagen, T. et al. Three-dimensional reconstitution of embryonic cardiomyocytes in a collagen matrix: a new heart muscle model system. FASEB J. 11, 683–694 (1997).
22.Zimmermann, W.H. et al. Three-dimensional engineered heart tissue from neonatal rat cardiac myocytes. Biotechnol. Bioeng. 68, 106–114 (2000).
23.Zimmermann, W.H. et al. Tissue engineering of a differentiated cardiac muscle construct. Circ. Res. 90, 223–230 (2002).
24.Carrier, R.L. et al. Cardiac tissue engineering: cell seeding, cultivation parameters, and tissue construct characterization. Biotechnol. Bioeng. 64, 580–589 (1999).
25.Leor, J. et al. Bioengineered cardiac grafts: A new approach to repair the infarcted myocardium? Circulation 102 Suppl. 3, III56–III61 (2000).
26.Li, R.K. et al. Survival and function of bioengineered cardiac grafts. Circulation 100 Suppl., II63–II69 (1999).
27.Zimmermann, W.H. et al. Cardiac grafting of engineered heart tissue in syngenic rats. Circulation 106, I151–I157 (2002).
28.Dhein, S., Muller, A., Gerwin, R. & Klaus, W. Comparative study on the proarrhythmic effects of some antiarrhythmic agents. Circulation 87, 617–630 (1993).
29.Menasche, P. et al. Autologous skeletal myoblast transplantation for severe postinfarction left ventricular dysfunction. J. Am. Coll. Cardiol. 41, 1078–1083 (2003).
30.Jackson, K.A. et al. Regeneration of ischemic cardiac muscle and vascular endothelium by adult stem cells. J. Clin. Invest. 107, 1395–1402 (2001).
4 5 8 |
VOLUME 12 [ NUMBER 4 [ APRIL 2006 NATURE MEDICINE |